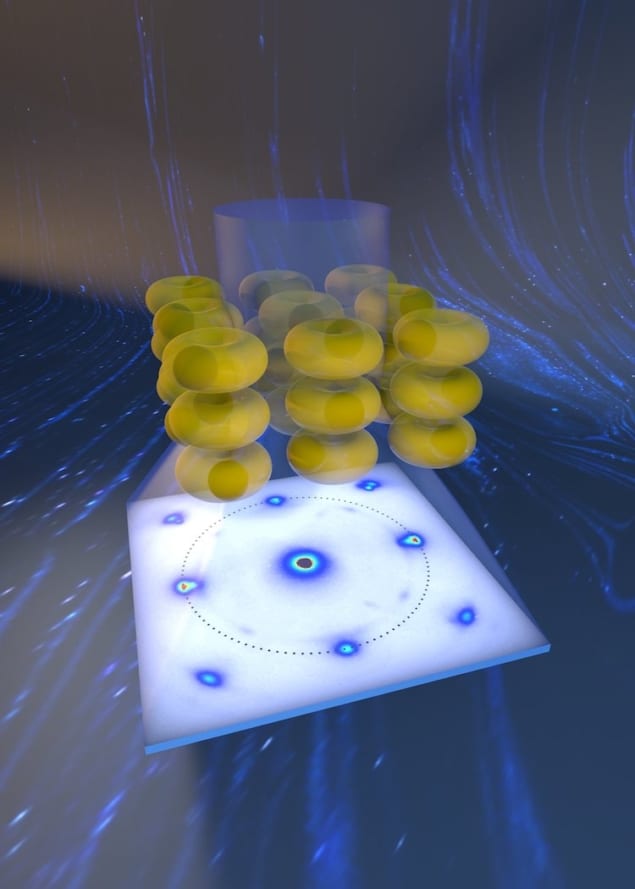
When a magnetic material is bombarded with short pulses of laser light, it loses its magnetism within femtoseconds (10–15 seconds). The spin, or angular momentum, of the electrons in the material thus disappears almost instantly. Yet all that angular momentum cannot simply be lost. It must be conserved – somewhere.
Thanks to new ultrafast electron diffraction experiments, researchers at the University of Konstanz in Germany have now found that this “lost” angular momentum is in fact transferred from the electrons to vibrations of the material’s crystal lattice within a few hundred femtoseconds. The finding could have important implications for magnetic data storage and for developments in spintronics, a technology that exploits electron spins to process information without using much power.
In a ferromagnetic material, magnetism occurs because the magnetic moments of the material’s constituent atoms align parallel to each other. The atoms and their electrons then act as elementary electromagnets, and the magnetic fields are produced mainly by the spin of the electrons.
Because an ultrashort laser pulse can rapidly destroy this alignment, some scientists have proposed using such pulses as an off switch for magnetization, thereby enabling ultra-rapid data processing at frequencies approaching those of light. Understanding this ultrafast demagnetization process is thus crucial for developing such applications as well as for better understanding the foundations of magnetism.
Where does the angular momentum go?
To find the lost angular momentum, Peter Baum and members of his team at Konstanz began by magnetizing nickel crystals in a specific direction, then demagnetizing them using a femtosecond laser pulse. They monitored what happened on femtosecond time scales by using ultrafast electron diffraction, a technique that can track structural changes in materials at the atomic level. They then analysed the diffraction patterns they obtained using computer simulations.
Just after demagnetization, collective lattice vibrations, or phonons, appear. These phonons are circularly polarized and are thus able to carry the missing angular momentum away from the electron spins. “The angular momentum goes directly into the phonons,” Baum explains. Team member Ulrich Nowak adds that their data “shows that the angular momentum of the electrons is transferred locally to the atoms of the crystal lattice on the same time scale on which the magnetic order of the crystal is lost”.
At first, only a few atoms move in circular orbits. Thanks to interactions with neighbouring atoms, however, this motion is then quickly transferred to all the other atoms. In the end, the entire crystal lattice uniformly oscillates in tiny circular obits. “On much slower time scales – nanoseconds, a million times slower than femtoseconds – the entire material starts to rotate as a whole,” Baum tells Physics World.
Atomic version of the “Einstein-de-Haas” effect
The work solves an old mystery in solid-state physics and proves experimentally that polarized lattice vibrations can indeed transport angular momentum quickly and effectively, Baum adds. The data also show that this atomic and ultrafast version of the “Einstein-de-Haas” effect, so-called after Albert Einstein and Wander Johannes de Haas measured it for macroscopic bodies more than 100 years ago, has an intermediate transitional step on the atomic scale.

Quantum microphone detects the presence of phonons
The team say the results might be exploited to control magnetic materials using laser light and therefore create more efficient alternatives to conventional electronics. “Unlike current electronic circuits, these devices would work with spin transport instead of charge transport, which would be significantly more energy-efficient,” Nowak explains. “By demonstrating that lattice vibrations can transport a spin, we open up a new, potentially promising path towards novel and particularly fast devices in spintronics.”
The researchers, who report their work in Nature, say they now plan to use their unique ultrafast electron diffraction measurements to study more complex magnetic materials and also the spatio-temporal transport of the circular phonons over barriers and into other materials. They are also advancing theory to make predictions that can help with the design of future applications. “There is a lot to do following our preliminary discovery,” Baum concludes.